Introduction to the CDF detector and the particles we observe
We briefly describe here the CDF detector and the types of particles we observe (which often come from interesting physics processes that we wish to study). Please also see:
- an introduction to the CDF experiment
- an introduction to the physics processes studied by the CDF experiment
- an introduction to the CDF Event Displays
- additional comments on the CDF experiment
See a photo of the CDF detector
INTRO--The CDF detector is a 100-ton complex detector which measured interesting
particles that came out of proton-antiproton collisions. Two intense beams
(about 10^14 to 10^15 particles each) of protons and antiprotons
met head-on in the middle of the CDF detector, and a few collisions occured
every time two bunches collided (every 120 nsec, or about 1/8-millionth
of a second). Most of the collisions were "glancing" collisions, with almost
all the energetic particles going along the beam in both directions, and only
a few low-energy particles going off at large angles (the ones detected by the
CDF detector--see COVERAGE below). A few of these glancing collisions per
second were recorded--mostly to be used as calibration of our detector, but also
for some physics measurements.
Out of the millions of collisions per second, only a few were "Hard" collisions--those producing energetic particles which went off at large angles (above 1-2 degrees from the beam, but sometimes even at 90 degrees to the incoming beam!). This happened when each of the energetic constituents of the proton and antiproton (quark or gluon) made a smashing hit and debris come off at large angles, like fireworks exploding. Sometimes, these smashing hits produced a short-lived particle, such as a W or Z boson; other times, just two jets of hadrons were formed by outgoing quarks or gluons. Something new also could happen in the collisions, such as the production of a new previously-unknown particle. Usually such a particle will decay rapidly--almost instantly--into other particles that we can observe. However, it is not enough for the new particle to be produced--to have a discovery we must show that the evidence of these decays is exceptional, and the observed signature could not come from any of the known processes (we call these known processes "background" to the "signal" of the new particle). Thus, we must measure the observable particles, "reconstruct" what happened in the event, and then show that the number of such events are well beyond what could be expected from backgrounds. This is the process that we went through in the discovery of the top quark.
Particles that we observe
What are the observable particles? We measure the particles that are produced at angles that will make them pass through our detector (see COVERAGE below). Only those particles that are charged under strong or electromagnetic interactions will be observed. Some particles that are neutral and do not interact in our detector will show up as "missing transverse momentum", by carrying off large transverse momentum which is not balanced by the other particles in the event which we observe (these non-interacting neutral particles include neutrinos, as well as possibly some new particles of similar persuasion). Conservation of momentum says that if we have a 40 GeV transverse momentum particle that goes to the left at 90 degrees, there must be one or more particles carrying a total of 40 GeV transverse momentum going off to the right. If we do not see any energetic particles to the right (and we have no hole in our coverage) then whatever carries 40 GeV transverse momentum off to the right must be one or more particles that we do not detect (such as a neutrino). In fact, if the 40 GeV particle to our left is an electron, or a muon, this is a perfect candidate for a W boson, an 80 GeV object that would decay (roughly 11 % of the time each) into electron + neutrino or muon + neutrino.
What are the characteristics of the particles that we observe? The particles we are able to observe can be categorized based on whether they are
- Electrically charged or neutral: Positions of charge particles can be measured accurately in our inner silicon (SVX) or outer (COT) tracker through the ionization left by the particle as it passes through (the ionized charge is amplified and turned into electronic signals that we record--allowing us to accurately measure the position of the track). Due to the strong magnetic field in the tracking detector, the degree of bending of the track in the end-on view tells us the momentum of the track--the straighter the track, the higher the momentum.
- Strongly charged--muons, electrons, neutrinos and photons do not undergo the strong interactions; almost all other particles (protons, neutrons, charged pions, Kaons, etc.) undergo the strong interaction.
- Showering particles in the electromagnetic detector: only electrons and photons (as well as photons from the decays of other particles such as the neutral pion) have a shower that leaves nearly all its energy in the front EM calorimetry.
- Electron (or positron)--a lepton of the first family (see additional information for more details of the Standard Model and the 3 families of leptons). A charged particle that produces an EM shower, and thus leaves nearly all its energy in the front EM calorimeter.
- Muon--a lepton of the second family. A charged particle that does not interact in the calorimetry--so will only leave a small amount of energy there (0.25 GeV in the "EM" and about 1.5 GeV in the "HAD" calorimetry). It will penetrate the iron and will leave a track in the Muon chambers. While the muon is unstable, its lifetime (2.2 microseconds) is long enough for essentially all of them to traverse our detector before decaying.
- Tau lepton--a lepton of the third family. This short-lived particle would
decay into many different states. In final states without either an electron
or a muon (2/3 of the time) the decay is dominantly to one charged pion
(along with neutral pi-zeros, and a neutrino), with a smaller fraction going into
three charged pions (+ neutral pi-zeros and a neutrino). The evidence
for tau lepton decays are narrow jets of pions with approximately a 2-to-1 ratio of
one charged pion (1-prong) to three charged pions (3-prongs).
We cannot determine that a particular jet comes from a tau-lepton decay--rather
we can estimate that in a sample of dozens (or hundreds) of events, there is a certain
proportion of tau leptons.
- Photon--a neutral particle that leaves all its energy in the EM calorimetry. Note that some photons come directly from the collision, while others come from the decays of such particles as neutral pions--it is a difficult task to determine what fraction of observed photons come from which source! This detective work is really difficult and requires dedicated detector components.
- Jet (quark or gluon)--a collimated bunch of charged and neutral particles usually with many charged particles--like streamers from a firework explosion.
- b-jet--a jet originating from a b-quark. This special type of jet contains a B-hadron among its decays. The B-hadron is special because it lives for a few hundreds of a nanoseconds, and thus will travel perhaps a few mm before decaying. We specially made very accurate detectors (see SVX below) very close to the beam and collision in order to measure the decaying tracks from such B-hadrons. Using these tracks we can determine that they arose from a "long-lived" particle. Identifying b-jets is crucial for identifying many physics processs, since many heavy objects prefer to decay into b-jets (e.g., the top quark decay product include a b-jet, and the Higgs boson preferentially decays into 2 b-quarks).
- c-jet--a jet originating from a c (charm) quark. This is similar to a b-jet, except that the charmed D-meson has a lower mass (less than 2 Gev) than the B-meson (5.2 GeV mass) and a slightly shorter lifetime. Thus, we are able to statistically differentiate between them (i.e., determine from a large sample--perhaps hundreds or more--the relative fraction of b-jets, c-jets, and light-quark jets.
- Neutrino--as mentioned above, the production of a neutrino is only inferred from the lack of momentum conservation in the event. Furthermore, we can only infer the transverse comoponent of this particle's momentum, not the component along the beam. Also, if there is more than one neutrino in an event, we only know the combined transverse momentum of all the neutrinos (or other neutral non-interacting particles) combined.
- "Soft particles"--in addition to the above energetic particles, each event typically has dozens of so-called "soft particles", often with no more than 1 GeV of energy, hich, unfortunately, make it more challenging to reconstruct the event correctly (especially in the tracking detector) by obscuring the high mementum tracks we are particularly interested in.
- Note that even if all these particles go through our coverage area,
a small fraction of the particles will not be recognized (we say
reconstructed) properly. This is a bias that we have to understand
before we can definitely say what we observe, and constitutes what
we call a "systematic uncertainty" in our final results (where any
measured quantity always has both systematic and statistical
uncertainties. So, we can never say that "We measure
the parameter xyz to be exactly 1.024"--we will always qualify the
results by saying something like this -- "We measure
the parameter xyz to be 1.024 +- 0.003 systematic and +- 0.004 statistical
uncertainties".
The statistical uncertainty comes from the fact that we have a finite number of observed events. For example, if you flip a coin (not a loaded one, please) 100 times, you may find that there are 48 heads and 52 tails--that's because 100 times give you a statistical error of 1/2 divided by square root of 100--so 5% statistical error-- so, the result which is 2% away from the correct one is entirely reasonable (you may also hear about statistical uncertaintly in political polling results--it is the same idea).
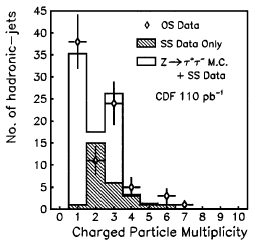
HOW WE MEASURE THESE PARTICLES
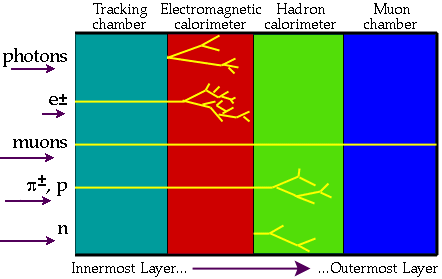
DETECTOR COMPONENTS--In order to measure the observable particles, we have several detector systems. Let's consider a particle coming off the collision at a large angle--say, 40-90 degrees from the beam. it will cross the following detector systems in order:
- SVX--SILICON VERTEX TRACKER (measuring charged particles only)--These are among the most accurate devices in measuring the position of charged particles, with an accuracy of about few tens of microns! Six layers of silicon provide an accurate determination of all tracks, and can identify tracks that do not point to the collision vertex-- and are thus candidates for being from the decay of a B-meson (or a charmed D-meson).
- COT--CENTRAL OUTER TRACKER (measuring charged particles only)--This tracker measures the position of a charged track accurately (~120 microns), and, based on the bend of the particle, also the momentum of the track.
- EM CALORIMETER--the front calorimetry consists of lead sheets sandwiched with scintillator (which measures the ionization from charged particles in the EM shower). The total amount of light observed is linearly correlated with the energy of the electron or photon--thus allowing us to measure the energy of the electron or photon. An energetic shower with no track pointing to it is a photon candidate (though it could be a neutral pion instead), while one that has a track with the same momentum would be a straightforward electron candidate.
- HAD CALORIMETER (the back calorimetry)--iron plates with scintillators sandwiched in between. Almost all EM showers are absorbed before reaching the HAD calorimetry. For hadrons (charged pions, kaons, and protons), most of the energy is left in the HAD calorimeter, with perhaps 10-30% in the EM calorimetry.
- IRON ABSORBER--placed behind the calorimetry and used to absorb all hadronic showers
- MUON CHAMBERS--if the charged particle makes it to the muon chamber, it is very unlikely to be a hadron (though there is one chance in perhaps 10,000)--and thus the particle is a good muon candidate, especially if the slope of the track is consistent with the momentum measured in the COT.
COVERAGE--You may have noticed that we have little or no detector in the direction of the beam--for very good reason. Since most glancing collisions produce lots of energetic particles along the beam direction, any detector there would be hit with so many particles that the detector would die. Also, since each bunch-crossing has millions of particles, we do not know which one came from the collision we are interested in. Furthermore, there is a vacuum beam pipe for the beam to go through (so that the beam does not get wasted by collision with air), and it is very difficult to install instruments next to vacuum (well, we do have some small detectors called Roman Pots, which stick onto the beam pipe to make some measurements).
So, our coverage starts at 1-2 degree from the beam, and covers all the "large" angle region, with progressively more sophisticated detectors. Coverage from about 30 degrees to 90 degrees (the Central region) is the most thorough--described above. Between 10 and 30 degrees, there is less tracking coverage (we reconstruct the track, but do not measure the momentum well), adequate calorimetry coverage, and muon coverage only down to 20 degrees.
WHAT WE RECORD--Out of the millions of collision per second, only a few
produce energetic particles at large angles (into our detector). All our
detectors--tracking chambers, calorimeters, etc--are instrumented to
record electronic information. The CDF detector has more than (500,000) such
channels of information! A small selected sample of these channels provide
very fast preliminary information (within microseconds) on what happened in
the collision, allowing us to determine whether the particular collision
is of sufficient interest to record all the information from the event
(we only record, or "trigger", about 50 events per second out of the millions
of collisions).
All the event information from the ~500,000 channels is digitized--with
channels that have null information (such as a scintillator that does
not have a signal) discarded. So, roughly 100,000 pieces of information
are recorded for every selected event (give or take a factor of 2--depending
on what's happening in the event--some events have 10-20 particles in our
detector, others have 50 or even 100 or more particles).
RECONSTRUCTION--In order to determine what the event is, we need to "reconstruct" the event--that is, measure all the particles that we observe, determine their characteristics, and then decide what type of event it is. For example, if we have an event with a well-measured 40 GeV electron candidate on one side, with nothing on the other side other than "soft" particles, this would be a candidate for a W boson decaying into an electron + neutrino.
PHYSICS ANALYSIS--Once we have collected a sample of a particular type of event (say, W-->e + nu candidates), we need to answer several questions. I give below a very brief overview--the whole list, even for one particular process, is extremely complicated and usually occupies one or more graduate student or post-doctoral fellow for many months or even years of burning the midnight oil--many of them have been known to work from morning to midnight for 6-7 days per week!
- What is the efficiency of our detector and trigger?
- What are the physics and detector backgrounds to the process we are studying?
- What is the result we derive from the events we obtain?
Now, for some comments on these issues--for a particular process we are studying
- What is the efficiency of our detector and trigger?
The trigger, which enables us to record only 50 or so events out of the millions of collision per second, could lose a small fraction of events, as well as having biases which will make us lose particular kinds of events.
Events will be lost due to selection criteria. In order to best study a process, we make selection criteria (cuts) so that only the "best" (i.e., those having the most advantageous ratio of signal to background) events are used for final analysis.
Confusion--some signal events would be lost due to tracks overlapping with other tracks in the event--and thus might be lost.
Some events may not be reconstructed properly.
We need to determine these efficiencies and biases in order to obtain any physics results--this involves many studies using data samples and Monte Carlo simulations (what-if scenarios in which we generate supposed signal or background events and pass them through a computer-simulated detector to see what would have shown up). - What are the physics and detector backgrounds to the process we are studying?
For each process we study, there are other processes which could give us events close to or even identical with the events from the desired process.
Some of these could be "artefacts"--events where we mismeasure some of the reconstructed particles due to noise or limited detector resolution
Others are events from other processes which mimic the signature we are studying--such as an electron candidate which arises from the overlap of a track with a photon energy deposit.
Still others are events with the correct signature, but come from other physics processes.
All these must be studied and the quantities determined. If we are looking for a new physics process, we must show that the number as well as the properties of events observed can not be accounted for by the "backgrounds" from known physics processes. - What is the result we derive from the events we obtain?
Once we have made a measurement, we need to provide the resulting value(s) of the measurement, as well as the systematic and statistical uncertainties of the measurement. - See Signal vs. Background for a more detailed discussion.
- Last modified
- 04/06/2022
- email Fermilab